The Technological Revolution that could Supercharge the World's Batteries
Silicon carbon batteries are a real game-changer. But they also have a big problem. To see why, you need to dive deep inside a battery. So let's do that.
Did you know that the battery inside your smartphone is literally changing shape - at this very moment?
Observe it for a moment. If it’s currently plugged into its charger you might notice an area of the phone getting hotter. That’s probably the battery. But what you can’t feel or see is that as well as heating up, the battery is swelling ever so slightly, gaining as much as 13 per cent in volume along the way. If the phone isn’t plugged in, that battery will be slowly and imperceptibly shrinking, all the way until it gets plugged in again.
This idea - that the devices we carry around with us are not inert but actually pulse; breathe, almost - is one of the more magical notions I encountered while researching Material World. In the sometimes spartan world of physics, every so often you find yourself colliding with poetry.
And the reason the shape of the cell changes comes back down to physics. Now is not the time to go through the full rigmarole of what the inside of a battery looks like (for more on that go here) but the main thing you need to know is that lithium ion batteries - which is to say most batteries inside our smartphones, our laptops, our drones and our electric vehicles - are all about the lithium ions.
Essentially, when the battery is charging, lithium ions (charged atoms) are shuffling across from the positive electrode, the cathode, to the anode, the negative electrode. In the book I likened this movement to the trudging of workers from one building to another. I’m not sure this is the best of all analogies but I find it helpful, since it underlines that somewhere beneath the surface, movement is happening.
When your smartphone is plugged in, electrically-charged lithium atoms are migrating across from the cathode where they live to nest inside the graphite matrix on the anode. When they reach the anode they sit alongside the carbon atoms. This is known as intercalation and working out how it could work in batteries was one of the breakthroughs for which Stanley Whittingham was eventually awarded a Nobel prize. Here’s a helpful diagram from Benchmark Mineral Intelligence.
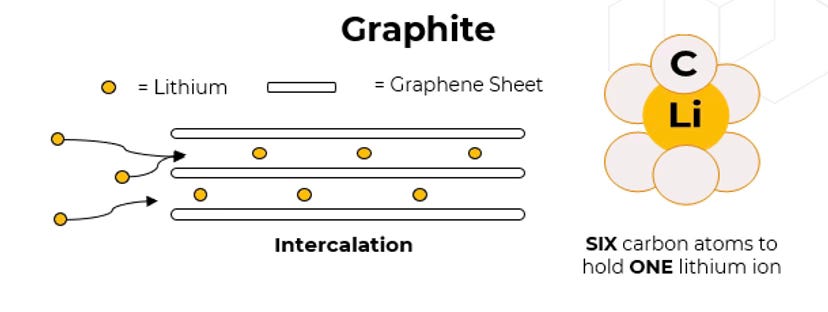
The battery is swelling because those graphite layers are being filled up with lithium ions. It’s heating up because a stampede is happening from one electrode to the other. Now, you don’t tend to see this because it’s not a massive swelling and, anyway, it’s happening under the casing of your phone and beneath the shell of the battery itself. But it’s still a physical transformation.
It’s worth keeping this in mind. When most people think about batteries they think of them as a kind of uniform little device but in reality there are two critical components inside them. The anode and the cathode (actually there’s more to it than that because there’s also the separator that keeps them apart and the electrolyte solution that lubricates the electrochemical reaction as those lithium ions commute from one electrode to another - but that’s for another day) are both equally important.
But invariably when you hear folks talking about batteries and why one kind is better than the other, they are usually banging on about the cathode. That’s the sexy part - the positive electrode. It’s the place where those lithium ions “live”, and by playing around with the cathode chemistry there are all sorts of clever things you can do to change the behaviour of the battery. You can add nickel, manganese and cobalt to increase its energy density. You can pair it with iron and phosphate to make it more stable and to improve its longevity.
The anode, the negative electrode, is generally ignored because it’s “just” graphite. But this is an enormous simplification. The way that graphite is produced and applied has a direct bearing on how good a battery it will be. You need the graphite to have the perfect molecular structure to allow as many lithium ions as possible to nest within it. You also need the graphite to allow those lithium ions to come and go as they please as quickly as they please.
So the anode, and how it’s made, is actually very, very important. To complicate matters further, there are different types of graphite, with different characteristics. There’s the natural graphite you mine out of the ground and the synthetic graphite you make from crude oil. Most battery anodes are a cocktail of the two, but the proportions of each can vary.
Broadly speaking, natural graphite is better at “holding on” to charge - so if you want a battery with decent resilience and long life, you want a generous proportion of natural graphite. Synthetic graphite, on the other hand, is particularly good at allowing those lithium ions in and out of the matrix, so is the key ingredient for fast charging batteries. If you have a smartphone with a fast-charging function - that’s partly a consequence of synthetic graphite (which is to say, petroleum-based carbon).
All of which is an incredibly long-winded way of building up to what I really wanted to talk about today - the most exciting thing happening in batteries today: silicon carbon anodes.
If you’re into new tech you might have heard of silicon carbon; it’s gradually surfacing in high-end smartphones, most notably the Honor Magic Pro range and the OnePlus 13. And it means those phones have batteries that are far more energy dense while remaining incredibly thin. Look: here’s a review from Marques Brownlee correctly pointing out that these batteries are the Next Big Thing we’re going to see a lot of in the coming year.
What’s exciting about these new batteries (and actually it turns out the ones you find in smartphones are only the tip of the iceberg) is that the anode is made not just of graphite (which is to say, carbon) but of a composite of graphite and silicon.
That matters when you think about what the anode is there to do - to provide a place for those lithium ions to nest when the battery is charged. The more hospitable you can make that nest, the better the battery will work. And silicon has properties that make it particularly attractive.
For one thing, silicon is much better at accommodating lithium ions than carbon. While it takes six carbon atoms to hold one lithium ion, a single silicon atom can hold four lithium ions. Or to put it another way, if your anode is made of graphite, six atoms of the anode will hold a single lithium ion; if your anode is made of silicon, six atoms will hold 28 lithium ions. That means you can theoretically hold far more lithium on a far smaller anode, which allows you to store much more energy in a comparable space.
Nor is that the only advantage. Silicon anodes can also enable far faster charging than in current batteries. Smartphones are an early adopter of the technology but these manufacturers are mostly using only a small fraction of silicon, nano-deposited onto the carbon.
But according to Benchmark, the potential could be even greater. Imagine an electric car you could charge in as little as 15 minutes (some producers even claim the technology will allow them to get to five minute charging times). All of a sudden, when you can charge an EV nearly as quickly as you can fill a petrol car, one of the last remaining objections to the technology drops away. So what’s the catch?
Well, first and most obviously, this is a nascent technology, so it’s considerably more expensive than standard graphite anodes. But the bigger obstacle comes back to physics.
Think back to all those lithium atoms clamouring to migrate across from cathode to anode, looking for somewhere to nest in the graphite matrix of your smartphone battery. Well, the reason graphite is typically used as an anode material is that those sheets of carbon are just very good at housing the lithium without too much degradation and too much swelling.
But the way it works with silicon in an anode is somewhat different (technically this is alloying rather than intercalating) and the upshot is silicon anodes swell far more than graphite anodes when they’re being charged. Indeed, they can swell up as much as 300 per cent more than a graphite anode. All of a sudden that magical breathing we talked about up at the top becomes a kind of all-encompassing wheezing that’s hard to accommodate inside a smartphone or an electric car.
As Rory McNulty of Benchmark says, the swelling: “can cause serious mechanical degradation (particle cracking, pulverisation, delamination from the current collector, etc.) which often results in poor cycle life and cell failure. Traditional silicon materials have had to find a balance between increased performance, but reduced cell lifetime."
These are not trivial challenges. And they’re part of the explanation for why it’s taken so long for scientists (who’ve long known about silicon’s potential as an anode) to come up with decent silicon anodes. They’ve had to engineer ways around the problem, either nano-sizing the silicon particles to a small enough size that gives them room to expand, or creating a kind of molecular “scaffold” within which they can do their thing.
In the very long run it’s possible we’ll have all-silicon anodes, and there are a few interesting companies working on precisely that, places like GDI and Nexeon not to mention many of the big battery firms.
But that swelling is a tricky challenge to manage. And so for the time being think of silicon as being something that’s kind of sprinkled into standard anodes to make them more effective. That’s what those new generation smartphone batteries typically have.
Alongside other big battery goals like solid state cells and lithium metal, silicon anodes promise to revolutionise batteries. But in the Material World, nothing is simple. Physical challenges are not always easily surmounted. Physics matters. And breakthroughs (when they come, which they often do) are the product of a lot of hard work and iteration.
PS I nearly forgot to add: if you’re wondering where the silicon in these batteries actually comes from, the short answer is from much the same place the silicon in silicon chips comes from. It’s mined out of the ground in the form of quartzite before being smelted into metallurgical silicon and then purified further. More on that in chapter three of Material World.
Hi Ed,
Fabulous technology explanation, but with one glaring omission.
At the very end, you mention GDI and Nexeon (fine firms and worthy of mention). But you bypassed the leader in silicon anode production and commercial traction, Group14 Technologies and their product SCC55.
In September, they commissioned the first EV-scale plant in the world for this stuff in Korea (JV with SK) at 2,000 metric tons/yr., 10 GWh. https://www.prnewswire.com/news-releases/group14-delivers-its-advanced-silicon-battery-material-to-over-100-customers-worldwide-from-an-ev-scale-factory-302249774.html
They are tracking to bring two more same-size plants live in Moses Lake, WA in 2025. https://www.youtube.com/watch?v=9RlH5-_P29A
And your worry about "swelling" has been solved.
Battery companies using SCC55 are showing cycle life above 1,000 while retaining 80% or better capacity.
Molicel is at 1,400 with their P50B https://www.molicel.com/inr-21700-p50b/ It can already be found lifting heavy loads in the North Sea. https://flyingbasket.com/blog/news-1/skylift-delivered-over-5-tons-of-wind-turbine-equipment-offshore-using-flyingbasket-heavy-lift-drones-25. And if you have 800,000 Euros to spare, how about the fastest car in the world? https://www.youtube.com/watch?v=WB3AHI40BFE
Sionic just announced their 100% silicon anode battery with 1,200 cycles. https://spectrum.ieee.org/silicon-anode-battery-2670396855
InoBat also is now visible with their high performance battery: https://www.inobat.eu/newsroom/tests-confirm-inobats-31ah-battery-offers-a-groundbreaking-solution-for-the-high-performance-mobility-markets/
And the two big dominos in consumer electronics may be close to falling via TDK's announcement, as well: https://www.firstpost.com/tech/iphone-battery-supplier-tdk-rolls-out-new-silicon-anode-batteries-to-keep-up-with-ai-devices-evs-13850273.html
Thanks for explaining this well enough for a chemistry-challenged person to see it clearly. (And to enjoy it all the way to the end of the article.) You're literally enlarging my known world.